Extractive Metallurgy Laboratory Guide
. 3.2k Downloads. Abstract This position paper introduces the reader to the concept of solvometallurgy, the term used to describe the extraction of metals from ores, industrial process residues, production scrap, and urban waste using non-aqueous solutions. Here, non-aqueous is not used to imply anhydrous, but rather a low water content. The unit operations are as follows: solvent leaching; separation of the residue; purification of the leach solution by non-aqueous solvent extraction or non-aqueous ion exchange; and metal recovery by precipitation or electrolysis in non-aqueous electrolytes.
Solvometallurgy is similar to hydrometallurgy in that both the branches of extractive metallurgy use low-temperature processes, but with solvometallurgy, there is no discrete water phase. Both branches use organic or inorganic solvents (excluding water in the case of solvometallurgy). However, for solvometallurgical processes to be sustainable, they must be based on green solvents, which means that all toxic or environmentally harmful solvents must be avoided. Solvometallurgy is complementary to pyrometallurgy and hydrometallurgy, but this new approach offers several advantages.
Metallurgical ContentBatch TestingContinuous TestingFlowsheet DesignSelecting Equipment SizesMill Design A successful search for and development of a new mineral deposit rests not only in its discovery but also in economical processing of the ore.
- Dec 18, 2015 - Solvent Extraction of Copper: An Extractive Metallurgy Exercise for Undergraduate Teaching Laboratories. Smellie†, Ross S.
- SRK Worldwide › Our Services › Extractive Metallurgy and Mineral Processing › Laboratory and Pilot Plant Testwork. From the laboratory through to pilot.
First, the consumption of water is very limited, and so the generation of wastewater can be avoided. Second, the leaching and solvent extraction can be combined to form a single step, resulting in more simplified process flow sheets.
Third, solvent leaching is often more selective than leaching with acidic aqueous solutions, leading to reduced acid consumption and fewer purification steps. Fourth, solvometallurgy is useful for the treatment of ores rich in soluble silica (e.g., eudialyte) because no silica gel is formed. In short, solvometallurgy is in a position to help develop the near-zero-waste metallurgical processes, and with levels of energy consumption that are much lower than those with the high-temperature processes. The Technology Readiness Level (TRL) of this emerging branch of extractive metallurgy is still low (TRL = 3–4), which is a disadvantage for short-term implementation, but offers a great opportunity for further research, development, and innovation.
Until a few centuries ago, mankind made use of only seven metals—copper, tin, iron, gold, silver, lead, and mercury—in pure form or in the form of alloys (e.g., bronze). In the early nineteenth century, metallic zinc began to be produced, although this metal had been used for centuries as a component of brass. All these metals were produced from high-grade ores by the high-temperature, pyrometallurgical processes.
However, once the rich ore deposits began to run out, there was no alternative but to start mining lower-grade ores, which could not be processed into a concentrate using the beneficiation methods that were employed at that time. This became a serious problem at the turn of the twentieth century and led to the development of mineral processing (e.g., froth flotation) and hydrometallurgical processing (e.g., leaching, ion exchange, and solvent extraction). Hydrometallurgy makes use of water as a solvent, and operates at much lower temperatures (20–200 °C) than pyrometallurgy (300 °C).
Hydrometallurgical processes were originally developed for the extraction of copper and gold, but the extraction of uranium from low-grade ores after World War II gave a boost to the development of hydrometallurgy. Hydrometallurgical flow sheets are now important for the extractive metallurgy of the rare-earth elements (REEs), zirconium, niobium, tantalum, gallium, indium, platinum-group metals (PGMs) zinc, and copper. The aluminum industry has also contributed to the development of hydrometallurgy; for example, the Bayer process, used to prepare pure alumina from bauxite ore, is the oldest example of a commercialized, high-pressure hydrometallurgical process. For the production of iron, pyrometallurgy is the dominant technique, whereas the flow sheets for the extraction of many other metals are based on a combination of pyrometallurgical and hydrometallurgical process steps.
For instance, after sintering and roasting the ore concentrate (i.e., a pyrometallurgical process), the calcine can be leached by an acid (i.e., a hydrometallurgical process). Hydrometallurgy is used only in the first parts of the flow sheet, and metal production is finalized quite often by pyrometallurgy.
As the demand for metals increases, the metallurgical industry has to rely increasingly on low-grade ores. In fact, industry is currently considering whether to recover metals from industrial process residues (e.g., bauxite residue, goethite, jarosite, phosphogypsum) or mine tailings, which were considered as waste until recently. Pyrometallurgical methods are often incapable of treating these residues in an economic way, because the metal concentrations are too low. Likewise, most hydrometallurgical processes are not very suitable for the recovery of metals from low-grade ores, tailings, or industrial residues with low concentrations of valuable metals, because leaching with aqueous solutions of acids suffers from a poor selectivity. This means that not only are the metals of interest dissolved, but also large parts of the matrix or gangue minerals go into solution, which leads to unnecessary acid consumption and generation of impure leachates. Alkaline leaching is more selective, but not all metals of interest can be solubilized by alkaline lixiviants. Furthermore, alkaline leaching causes the dissolution of large amounts of silica.
The processing of urban waste (e.g., waste electric and electronic equipment, WEEE) by hydrometallurgical processes has some specific issues. For instance, the dissolution of metals and alloys from scrap through the use of acids will form large volumes of flammable hydrogen gas, while the dissolution of semiconductor materials such as gallium arsenide can generate highly toxic arsine gas. The recovery of metals from mine tailings, industrial process residues, and urban waste helps to close the materials loop and to establish a circular economy.
Fig. 1 Zero-waste approach to metal-containing waste streams (Color figure online) In cases where pyrometallurgical or hydrometallurgical processes fail to efficiently extract metal values from low-grade ores, mine tailings, industrial process residues, or urban waste, we could turn to solvometallurgy, which constitutes a branch of extractive metallurgy that is complementary to both pyrometallurgy and hydrometallurgy. Solvometallurgy makes use of processes involving the non-aqueous solvents. These non-aqueous solvents can be molecular organic solvents, ionic liquids, deep-eutectic solvents (DESs), but also the inorganic solvents such as liquefied ammonia, concentrated sulfuric acid, or supercritical carbon dioxide.
High-temperature molten salts and liquid metals are excluded if they require temperatures above 300 °C. Non-aqueous solvents do not imply anhydrous conditions, but rather a low water content. Some solvometallurgical processes must take place in the absence of water, while for other processes, a low water content is required. Strictly speaking, conventional solvent extraction, which is based on the preferential distribution of metal ions between an aqueous phase and a water-immiscible organic phase, is not a solvometallurgical process because of the presence of an aqueous phase.
The original concept of solvometallurgy dates from the late 1940s and early 1950s when researchers in the United States were developing processes to recover uranium from the uranium ores through leaching with organic mixtures such as acetone-HCl or alkylphosphoric acids in kerosene ,. At that time, the term “ lyometallurgy” was introduced to describe solvent-based leaching processes, derived from the Greek word lyein, meaning to loosen or to dissolve. Other suggested terms included “ anhydrometallurgy” and the contradictory “ non- aqueous hydrometallurgy.” Later, solvometallurgical methods were used for the recovery of copper from chrysocolla, a largely amorphous copper silicate ,.
It was also realized that solvometallurgy could be useful for the reprocessing of spent nuclear fuel. Sastri reviewed the possibility of using organic solvents and chelating agents for the processing of ores. In 1985, in his book on ion solvation, Marcus used the term “ solvometallurgy” to describe the extension of hydrometallurgy to solvents other than water. Russian researchers also applied the solvometallurgical methods to recover metals from complex silica-rich ores (e.g., eudialyte), urban waste, and pellets of nuclear fuel ,. In recent years, there has been progress in the use of ionic liquids and deep-eutectic solvents in metallurgical processes, ranging from leaching, to solvent extraction, and the electrodeposition of metals ,.
The term “ ionometallurgy” was coined for metallurgical processes involving the ionic solvents , but ionic-liquid processes are solvometallurgical as well. Although we could consider hydrometallurgy to be part of solvometallurgy (since water is a solvent), we want to make a clear distinction between hydrometallurgy (based on water as solvent) and solvometallurgy (based on non-aqueous solvents).
In this paper, we describe the concept of solvometallurgy and its potential applications in extractive metallurgy. An overview of solvometallurgical unit processes is provided, and ten reasons are presented to highlight why solvometallurgy is a useful complement to hydrometallurgy and pyrometallurgy. Because we realize that several hurdles must be overcome before solvometallurgy will become commonplace in extractive metallurgy, the main challenges facing this new approach are discussed.
Selected examples of solvometallurgical processes reported in the scientific literature and the patent literature are described. Attention is paid to the selection and disposal of the solvents, because the use of green solvents is a must for the development of sustainable solvometallurgical processes. Solvometallurgical Unit Processes. Most of the unit processes in solvometallurgy are very similar to those in hydrometallurgy, with the main difference being that the water is replaced by a non-aqueous solvent. Table shows a comparison of the hydrometallurgical and solvometallurgical processes. Some solvometallurgical processes make use of solvent–water mixtures, but the solvent concentration is assumed to be at least 50 vol%, because otherwise the phase should be considered as an aqueous phase with added solvent, rather than as a non-aqueous phase.
It is important to note that the word solvent can have different meanings in solvometallurgy, and therefore the intended meaning must be derived from the context. The most general meaning of solvent is that of an organic phase, as opposed to an aqueous phase in hydrometallurgy. A solvent can contain different components, such as the extractant, the diluent, and dissolved acids. The diluent is an organic solvent, the main purpose of which is to obtain an organic phase with a reasonably low viscosity, if the extractant is a viscous liquid. According to the more strict definition, the term solvent is used to indicate the diluent. Another approach to solvent leaching is the use of water-immiscible (nonpolar) organic solvents with dissolved extractants.
Here, the lixiviant is very similar to the organic phase used in solvent-extraction processes ,. If an acidic extractant is used, such as a dialkylphosphoric acid (e.g., D2EHPA) or a carboxylic acid (e.g., Versatic acid), the organic phase can be applied directly for the solvent leaching of a solid material, without the need to add a mineral acid. Higher selectivities for leaching can be achieved by using solutions of chelating extractants as lixiviants. Some examples include α-hydroxyoximes (e.g. LIX ® 63), β-hydroxyaryloximes (e.g.
LIX ® 65 N), β-diketones (e.g. LIX ® 54), and 8-hydroxyquinolines (e.g.
Kelex ® 100). In Fig., the structures of some commonly used extractants are shown. Solvent leaching has a number of routes (Fig. The organic extractant phase can be brought into direct contact with the solid material; this approach is called “ direct solid leaching.” The solid can be a crushed and grinded ore, but it can also be a fine-grained industrial process residue, or even a solid that is obtained by the dehydration of aqueous leachates or by precipitation from aqueous leachates.
After the solvent leaching, the liquid organic phase and the solid residue can be separated by filtration, decantation, or centrifugation, followed by washing of the solid residue with the diluent or with another organic solvent to reduce the amount of solvent retained by the solid particles. Alternatively, the solid material can be brought into contact with both a concentrated mineral acid (typically concentrated H 2SO 4) and the organic phase. The mineral acid acts as a solubilizing agent.
It is also possible to digest the ore first with the mineral acid (i.e., an acid cure), before the damp solid is brought into contact with the organic phase. This approach, with the addition of a concentrated acid and an organic extracting phase to the solid material, is called “ non- aqueous-slurry leaching,” because the acid and the solid form a slurry with a low water content. After leaching, the liquid organic phase and the solid residue can be separated by filtration, decantation, or centrifugation, followed by washing of the solid residue with a solvent, just as in the case of direct solid leaching. “ Non-aqueous-slurry leaching” should not be confused with “ aqueous-slurry solvent extraction,” which is also known as “ solvent- in- pulp extraction”. In the latter approach, an organic extraction phase is brought into contact with a slurry of the solid material and an aqueous phase. After leaching, the organic phase can be separated from the aqueous phase and the solid residue after phase disengagement by simple decantation.
Extractive Metallurgy Laboratory Guidelines
However, an aqueous raffinate is also obtained, which then needs to be treated further. “ Aqueous-slurry solvent extraction” is not considered as a solvometallurgical method, because of the presence of a discrete aqueous phase. It is important to note that in the three approaches, i.e., direct solid leaching, non-aqueous-slurry leaching, and aqueous-slurry solvent extraction, the metallurgical unit operations of leaching and solvent extraction are combined into a single step. This is a form of process intensification, compared with the traditional approach, where leaching and solvent extraction are carried out as two separate steps.
After solvent leaching, both direct solid leaching and non-aqueous-slurry leaching give an organic-pregnant leach solution in which the metals of interest are dissolved. The metals can then be recovered from this organic phase using different approaches, depending on the polarity of the organic phase.
If the organic phase contains a solvent with low polarity, the organic phase can be treated in the same way as a loaded organic phase in solvent extraction, using a scrubbing step with an aqueous solution to remove the co-dissolved impurities and a stripping step to recover the valuable metals. The stripping step can be carried out with an aqueous stripping solution or with a stripping reagent (for precipitation stripping). In principle, the aqueous phase can be replaced by a polar organic phase, which is immiscible with the less-polar phase. If the solvent leaching is performed using a polar organic phase, the metals can be recovered by precipitation, non-aqueous ion exchange, the use of a solid adsorbent (e.g., activated charcoal), or by non-aqueous solvent extraction with an immiscible organic phase.
Fig. 3 Comparison of different approaches to solvent leaching involving hydrometallurgical leaching/extraction methods. Direct solid leaching and non-aqueous-slurry solvent leaching are solvometallurgical processes, while aqueous-slurry solvent leaching is not. Figure adapted from Ref.
(Color figure online) Non-Aqueous Solvent Extraction Solvent extraction (SX) is a very important technique in hydrometallurgy for the separation and purification of metals such as cobalt, nickel, copper, zinc, uranium, REEs, and PGMs. In conventional solvent extraction, the metal ions are distributed between an aqueous phase and a water-immiscible organic phase, in general with the aid of an extractant (also called the extracting agent).
Because the various metal ions distribute differently between the aqueous and organic phases, mixtures of metal ions can be separated by solvent extraction. It is clear that an aqueous phase is present in all the hydrometallurgical solvent-extraction processes. This could give the impression that all the solvent-extraction systems must involve an aqueous phase. However, this is not correct: all that is required are two mutually immiscible liquid phases. Solvent-extraction systems can also consist of (1) two mutually immiscible molten salts, (2) a molten salt and a molten metal, (3) a molten salt and an organic solvent, or (4) two mutually immiscible organic solvents. An important point with respect to solvometallurgy is the possibility to perform solvent extraction with two immiscible molecular organic solvents, two immiscible ionic liquids, or combinations thereof (Fig. It is obvious that we cannot speak about the aqueous phase and the organic phase, as is the case with conventional solvent extraction.
It is better to use the terms more-polar phase and less-polar phase. The solvent pairs for non-aqueous solvent extraction need to fulfill the following requirements: (1) ability to form two immiscible phases and have low mutual solubility of the two solvents; (2) exhibition of rapid phase separation after mixing; (3) demonstration of good solubility of the extractant in the less-polar phase and poor solubility of the extractant in the more-polar phase; and (4) demonstration of good solubility of the to-be-extracted metal salts in the more-polar phase and good solubility of the extracted metal complexes in the less-polar phase. The first condition is the most difficult to fulfill because most organic solvents are mutually miscible. However, several guidelines are available for the initial selection of solvent pairs, such as reported miscibility data , , the Hildebrand solubility parameter δ for solvents , and the mixotropic series ,. It is clear that the solvent plays a crucial role in solvometallurgy and that careful solvent selection is essential. However, the selection of the best solvent for a solvometallurgical process is not only based on performance. Stability, the possibility to recycle the solvent, and economics, because environmental impact, safety, and toxicity must also be considered.
The sustainability of a solvometallurgical process is closely related to the solvent on which the process is based. A sustainable solvometallurgical process cannot make use of harmful and dangerous solvents. Such solvents must be avoided, even if they would perform well in a specific leaching or extraction process.
A large number of organic solvents are commercially available at a reasonable cost, but not all of these solvents are useful in solvometallurgical processes. Table lists the most relevant organic solvents for solvometallurgy, divided into three classes: (1) preferred, (2) usable and (3) undesirable, according to the Pfizer “traffic light” solvent-selection guide. If possible, the solvents listed in the first column of Table should be used (= preferred solvents). If no suitable solvent is found in the first column, a solvent listed in the second column can be tried (= usable solvents). However, solvents listed in the third column of Table (= undesirable solvents) must be strictly avoided for the development of sustainable solvometallurgical processes. These solvents either have a very low flash point, easily form explosive peroxides, are ozone-depleting substances, have other environmental issues, they are toxic or they are carcinogenic.
There is little ambiguity about the list of solvents in the third column, in the sense that all these solvents are problematic. The division of solvents among the first two columns is, however, more arbitrary, because the sustainable character of a solvent is determined by several criteria, including workers’ safety, process safety, and environmental concerns. For more information on solvent-selection guides, the reader is referred to the literature ,. It is important to realize that these solvent-selection guides were drawn up for the chemical and pharmaceutical industries and not for extractive metallurgy. Nevertheless, many conclusions have general validity.GTL = gas-to-liquid Ionic liquids and deep-eutectic solvents are not listed in Table because the properties of these solvents are very much dependent on the composition. The compositional range here is too wide to draw any general conclusions. However, these solvents are often considered as green solvents because of their nonvolatility, low flammability, and, in general, low toxicity.
Volatile solvents might appear to be very useful because they can be recycled by distillation. However, they contribute a great deal to smog formation and present health risks through inhalation. Many volatile solvents have a low flash point, are flammable, and can even form explosive mixtures with air.
If possible, distillation should be avoided as a recycling method for organic solvents because this technique is energy intensive and requires the use of volatile solvents. It is, therefore, recommended to explore nonthermal techniques for solvent recuperation rather than distillation. An example of such a technique is organic solvent nanofiltration (OSN), also known as solvent-resistant nanofiltration (SRNF), which is a pressure-driven membrane technique. The environmental impact of a solvent requires more attention when the solvent is used in a system that is open to the environment, rather than a fully closed system. A solvent used in an open system should be nonvolatile and have a low toxicity. In the case of solvents used for leaching in open systems (e.g., for heap leaching), the biodegradability, i.e., the breakdown of chemical compounds by microorganisms, of the solvent is an asset.
Water-soluble esters, ketones, alcohols, and glycol ethers are readily biodegradable, while hydrocarbon solvents often have a much slower biodegradability due to their poor solubility in water, but there are differences in the degradation rate for the different classes of hydrocarbons. The order of susceptibility to biodegradation is: n-alkanes branched alkanes low-molecular-weight aromatics cyclic alkanes. Chlorinated and fluorinated solvents are, in general, poorly degradable. Several ionic liquids have a poor biodegradability, although other types of ionic liquids have been specifically designed to be biodegradable. On the other hand, biodiesel is an easily biodegradable and nontoxic solvent. Biodiesel is a mixture of mono-alkyl esters of long-chain fatty acids derived from vegetable oils or animal fats ,. It has been successfully tested as a diluent in solvent-extraction studies ,.
Extractive Metallurgy Laboratory Exercises
Soybean oil methyl esters have also been investigated as a diluent for solvent-extraction systems. Soybean oil consists of a mixture of fatty acids, with the vast majority of the fatty-acid component being linoleic acid, an 18-carbon, long-chain fatty acid with double bonds at carbon atoms 9 and 12.
Glycerol is a side product formed during the production of biodiesel, and it is a good solvent for many metal salts. It can also be used as a starting product for the production of other green solvents such as alkyl glycerol ethers.
Methanesulfonic acid (MSA) is an example of a strongly acidic organic solvent that can be considered as a green solvent. Methanesulfonic acid has a low toxicity, is readily biodegradable, and is part of the natural sulfur cycle. In general, metal methanesulfonate salts have a very high solubility in water so that they are often considered as a metal source in the preparation of aqueous electrolytes for metal deposition.
A new class of solvents that shows great promise for applications in solvometallurgy is solvents prepared by gas-to-liquids (GTL) technology. GTL solvents are prepared from natural gas using the Fischer–Tropsch process. The GTL process consists of three stages. In the first stage (syngas reforming), the natural gas is partially oxidized to create synthesis gas (syngas), a mixture of hydrogen and carbon monoxide, which is then purified. In the second stage (the Fischer–Tropsch reaction), the syngas is converted by a catalyst to a long-chain, heavy paraffinic liquid, the Fischer–Tropsch wax, which consists of a mixture of many compounds. In the third stage (product upgrading), the oxygen-containing products and alkenes are hydrogenated to alkanes. Through hydrocracking and isomerization, the paraffins are converted into smaller alkanes with a very characteristic branching pattern.
Using distillation, fractions with a different distillation range are obtained. The GTL solvents consist mostly of iso-paraffins and normal paraffins, with only very small amounts of cyclic paraffins.
They contain small amounts of impurities such as sulfur, olefins, and polycyclic aromatics. They are almost odorless, due to their low aromatic and naphthenic content.
Shell offers a range of commercial GTL solvents with different distillation ranges (GS190, GS215, GS250, GS270, GS310). The solvents with the higher distillation ranges have the lowest volatility, but they also have a higher viscosity (although it must still be considered as a relatively low viscosity).
A very interesting property of these solvents is their good biodegradability ,. GTL solvents were found to exhibit more than 60% biodegradation over the required 28-day test period, with the degradation continuing after the 28 days toward complete degradation.
Therefore, they are considered to be readily biodegradable according to the OECD 301F guideline. GTL solvents have better biodegradation scores than comparable petroleum-derived solvents because of the lower proportions of aromatics and/or naphthenics in the GTL solvents.
Organic solvents tend to degrade over time. In general, this is a disadvantage compared with water. In many cases, organic impurities can be removed by a washing step with water or with an organic solvent. In other cases, solvents can be purified by distillation, even though this is an energy-intensive process. This purification process cannot be repeated endlessly, and at any given moment, the solvent has to be replaced by a fresh batch of solvent. At the end of the lifetime of the solvent, safe disposal is required.
The best approach is to burn the spent solvent, with heat recuperation. The disposal by incineration is one of the reasons why halogenated solvents must be avoided. Halogenated solvents cannot readily be handled in most incinerators. Although inorganic solvents (with the exclusion of water, of course) can in principle be used for solvometallurgical processes, inorganic solvents are generally of little use in this context because they are too reactive, too dangerous, too toxic or too difficult to handle. Examples include liquid ammonia, liquid sulfur dioxide, anhydrous hydrogen fluoride, thionyl chloride, sulfuryl chloride, phosphoryl chloride, dinitrogen tetroxide, and antimony trichloride. Concentrated sulfuric acid is an inorganic solvent, but it is not used in solvometallurgy as a solvent, but rather for ore acidification (acid curing of ores).
Supercritical CO 2 could find applications in the field of non-aqueous solvent extraction and solvent leaching ,. Of particular interest are the promising combinations of supercritical CO 2 with TBP-HNO 3 and supercritical CO 2 with fluorinated β-diketones as solvents for direct solid leaching ,. CO 2 becomes a supercritical fluid at its critical temperature (31.10 °C) and critical pressure (72.8 atm). Any supercritical fluid expands to fill a container, but it has a density that is similar to that of a liquid. Ionic Liquids (ILs) and Deep-Eutectic Solvents (DESs).
Ionic liquids (ILs) are a special class of solvents that consist entirely of ions ,. This differentiates ionic liquids from both conventional organic solvents, which consist of neutral molecules, and from salt solutions, which consist of a mixture of neutral molecules and ions. Many researchers define ionic liquids as organic salts with a melting point below 100 °C, but this definition is too restrictive, and the threshold of 100 °C (the normal boiling point of water) is arbitrary. There are many examples of ionic liquids with melting points above 100 °C that have properties very similar to those of low-melting ionic liquids.
Of course, low melting points are an advantage, and the room- temperature ionic liquids (RTILs) are preferred. The typical cations in ionic liquids are organic cations with one or more alkyl chains. Examples include 1-alkyl-3-methylimidazolium, N-alkylpyridinium, N-alkyl- N-methylpyrrolidinium, tetraalkylammonium, and tetraalkylphosphonium cations. Anions can be inorganic, such as chloride, bromide, iodide, thiocyanate, tetrafluoroborate, hexafluorophosphate, bis(trifluoromethylsulfonyl)imide (=bistriflimide), or organic, such as acetate or benzoate.
By combining these cations and anions, a wide range of ionic liquids can be obtained (Fig. A special type of ionic liquids is the “liquid metal salts” that have a metal-containing cation. Molten-salt hydrates also belong to this class of ionic liquids. In molten-salt hydrates, all the water molecules are strongly coordinated to the metal ions so that there are no free water molecules in the melt.
Examples include CaCl 26H 2O, or Mn(NO 3) 26H 2O. Also worth mentioning are the functionalized ionic liquids in which a functional group is covalently attached to the ionic liquid cation. In the past, these ionic liquids were often called “task-specific ionic liquids”.
However, “functionalized ionic liquids” is nowadays the preferred term. A well-known example of a functionalized ionic liquid is betainium bis(trifluoromethylsulfonyl)imide, HbetTf 2N, which contains a carboxylic acid functional group (Fig. Fig. 6 Structure of the ionic liquid HbetTf 2N Although the physicochemical properties of ionic liquids are very dependent on the chemical composition of the ionic liquid, we can also make some generalizations. First of all, many ionic liquids have a nonmeasurable vapor pressure at room temperature, although some ionic liquids can be distilled in a high vacuum at high temperatures. Ionic liquids often have a very wide liquidus range; it is not uncommon to have ionic liquids that are fluid over a range of more than 250 °C, which is much wider than the 100 °C range of water.
Ionic liquids are intrinsic electrical conductors, and they can have an electrochemical window of more than 4 V (the electrochemical window of water is just 1.23 V). Ionic liquids are good solvents for many classes of inorganic and organic compounds. Compared with molecular organic solvents, ionic liquids have a much higher viscosity, which can hamper mass transport and slow down the rate of chemical processes. However, the viscosity of ionic liquids reduces dramatically upon heating or the addition of a small amount of a molecular co-solvent. A drawback of many ionic liquids is their high price, and this has hindered the development of ionic-liquid processes on an industrial scale. A cheap alternative to ionic liquids are the deep- eutectic solvents (DESs) , , of which there are many different types. Most of them are mixtures of choline chloride and a hydrogen-bond donor (urea, ethylene glycol, malonic acid) or mixtures of choline chloride with a hydrated metal salt.
A typical feature of a DES is that it has a much lower melting point than each of its individual components. Popular DESs are those obtained by mixing chloride and urea in a 1:2 molar ratio, or choline chloride and ethylene glycol in a 1:2 molar ratio. These solvents are liquid at room temperature.
Choline chloride is produced on a large scale and is used as chicken feed. Due to the low cost of DESs, it is much easier to use them on a large scale than ionic liquids. Although DESs often have a significant water content, their properties are generally different from those of aqueous solutions. As mentioned before, hard chrome layers can only be electrodeposited from aqueous electrolytes using toxic chromium(VI) salts, whereas the metal can be deposited from DESs containing the much-less-toxic chromium(III) salt.
In fact, a DES electrolyte for chromium deposition can be prepared by simply mixing CrCl 36H 2O and choline chloride , or by mixing CrCl 36H 2O and urea. Although deep-eutectic solvents cannot be considered as ionic liquids, as they are mixtures of ions and smaller or larger amounts of neutral molecules, their properties are very similar to those of conventional ionic liquids. For this reason, they are often described as ionic-liquid-like solvents, or just as ionic liquids. Due to their water content, the electrochemical window of DESs is smaller than that of conventional ionic liquids. The most obvious applications for ionic liquids and deep-eutectic solvents in solvometallurgy are in the electrodeposition of metals from non-aqueous electrolytes.
Commonly used ionic liquids for electrodeposition are 1-butyl-3-methylimidazolium bis(trifluoromethylsulfonyl)imide, C 4mimTf 2N, or N-butyl- N-methylpyrrolidinium bis(trifluoromethylsulfonyl)imide, BMPTf 2N, as well as several deep-eutectic solvents. Other applications of these ionic solvents in solvometallurgy are as solvents for solvent leaching and in non-aqueous solvent extraction. As mentioned in the introduction, the term “ ionometallurgy” is used for solvometallurgical processes based on ionic liquids or deep-eutectic solvents. Ten Good Reasons to Use Solvometallurgy. Very limited water consumption Reason 2.
Reduced energy consumption Reason 3. Process intensification Reason 4. Reduced consumption of acids Reason 5. Higher selectivity for leaching Reason 6.
Fewer problems with silica gel formation Reason 7. Suitable for the treatment of low-grade ores, mine tailings, and industrial process residues Reason 8. Useful for the treatment of urban waste Reason 9. Compatibility with bioleaching Reason 10. New separation processes Reason 1. Very Limited Water Consumption Because no bulk aqueous phase is present in a solvometallurgical process, the consumption of water can be limited to a strict minimum. In some solvometallurgical processes, a small amount of water is essential, but the water volumes are always small compared to the volumes of organic solvents, and in most cases no discrete aqueous phase is present.
If no water is consumed in a solvometallurgical process, it also implies that no wastewater is generated, and that there are no issues with wastewater treatment. Solvometallurgical processes have the potential to recover metals from ore deposits in arid areas, where it is difficult to guarantee a sufficient supply of process water.
This is very relevant for exploiting copper mines in the Atacama Desert in Chile. Likewise, solvometallurgical processes can also be operated in (mobile) stand-alone units that can be used to recover metals from small ore deposits at remote locations. Reduced Energy Consumption Solvometallurgical processes offer the opportunity to treat, at ambient temperatures, ores that otherwise have to be heated to high temperatures to convert them to oxides before they can be further processed.
A good example is the calcination of carbonate ores. By means of chlorine gas dissolved in an organic solvent, it is possible to chlorinate ores at ambient temperatures, instead of using chlorination or carbochlorination at high temperatures. The possibility to work at room temperature or at slightly elevated temperatures results in a considerably reduced energy consumption compared with pyrometallurgical processes, which are typically carried out at temperatures above 300 °C.
In general, organic solvents have a lower heat of vaporization than water, so that less energy is required to distil the organic solvent (for solvent recycling) than to distil the same volume of water. However, as mentioned elsewhere in this paper, the use of volatile organic solvents and distillation must be avoided, whenever possible. Process Intensification Solvometallurgy allows for process intensification, because different unit operations (e.g., leaching and solvent extraction) can be combined, and this reduces the number of process steps. A combination of mechanical activation (e.g., by ball milling) and solvometallurgy allows the treatment of resistant ores such as columbite, tantalite, and pyrochlore under much milder conditions (ambient temperatures, no HF) with respect to traditional metallurgical methods. Reduced Consumption of Acids Even if acid curing of the ore is used as a pretreatment step prior to solvent leaching, the total acid consumption can be much lower than that in the case of conventional leaching. This is because a larger excess of acid is often used in conventional acid leaching and because protons can be provided by the extractant in the case of solvent leaching with acidic extractants. Hydrometallurgical processes with acid leaching are not very compatible with carbonate-rich ores and especially not with those rich in calcite or dolomite, because the dissolution of the carbonate gangue material consumes large amounts of acids.
This is relevant if the minerals in a carbonate matrix cannot be separated from the gangue by flotation, e.g., in the case of the uranium ore carnotite. By solvent leaching the ore minerals, the carbonate gangue materials can be left largely untouched. Because solvent leaching is more selective than leaching with aqueous solutions of mineral acids, it is possible to avoid the co-dissolution of unwanted metals such as iron, which also leads to a reduction in the consumption of acids (see also Reason 5). Higher Selectivity for Leaching In general, leaching with aqueous solutions of mineral acids (H 2SO 4, HNO 3, HCl) suffers from low selectivity. Besides the targeted metals, many unwanted metals are co-dissolved. This poor selectivity leads not only to a higher consumption of acids (see Reason 4), but also to extra process steps for the removal of impurities from the leachate. Solvent leaching can, in principle, offer a higher selectivity than leaching with aqueous solutions of acids, especially if chelating extractants are used, such as the LIX ® family of extractants.
Fewer Problems with Silica Gel Formation For the recovery of technological metals such as rare earths, zirconium, tantalum, or niobium, we are forced to rely more and more on ores with a complex composition and a high content of soluble silica, because of the depletion of ores with a simpler chemical composition. A good example is eudialyte, Na 15Ca 6(Fe,Mn) 3Zr 3(Si,Nb,REE)Si 25O 73(OH,Cl,H 2O) 5. The leaching of such minerals with mineral acids dissolves the silica as silicic acid. This dissolved silica is gradually transformed by polymerization into colloidal silica, and these colloids can aggregate to form a gel. Gel formation in a leachate makes the filtration for solid–liquid separation impossible.
Dissolved silica can also interfere with solvent extraction, because it causes the formation of extremely stable emulsions during the mixing of the aqueous and the organic phases. These emulsions can be stable for weeks or even months. Silica colloids can also block the pores in ion-exchange resins. Dissolved silica is not only a problem in the case of acidic leaching; it is even more so in the case of alkaline leaching, because silica has a higher solubility in alkaline solutions.
Because water is required for the formation of silicic acid, it is evident that the leaching of silica-rich ores with non-aqueous solvents leads to much lower solubilization of the silica and hence to fewer problems with silica gel formation. Suitable for the Treatment of Low-Grade Ores, Mine Tailings, and Industrial Process Residues As mentioned in the introduction, the depletion of high-grade ores with a simple composition necessitates the shift to low-grade ores, mine tailings, and industrial process residues that contain only low concentrations of valuable metals and often have a complex composition with several mineral phases. Conventional leaching with aqueous solutions of mineral acids lacks selectivity, leading to the consumption of large amounts of acid and the co-dissolution of unwanted impurities. In particular, the co-dissolution of the matrix is a major problem. Solvometallurgical processes are suitable for the recovery of metals from low-grade ores (e.g., nickel laterites), tailings from primary mining, or industrial process residues (e.g., bauxite residue, goethite, or jarosite) because of their intrinsic higher selectivity (see also Reasons 4 and 5). Useful for the Treatment of Urban Waste In ore deposits, the metals typically occur in complex polymetallic sulfide or oxide ores, whereas the metals of the urban mine are typically present in the form of alloys. Examples are the rare-earth permanent magnets, which consist of neodymium-iron-boron or samarium-cobalt alloys.
The direct reuse of these alloys is often not possible, and the mixture of constituting metals needs to be separated into the individual elements. In hydrometallurgical recycling methods, the alloys are dissolved in aqueous solutions of strong mineral acids (H 2SO 4, HCl, HNO 3). This step can be unsafe because of the formation of flammable hydrogen gas during the metal dissolution. In the case of arsenic-containing alloys (e.g., GaAs), there is a danger of formation of highly toxic arsine gas (AsH 3). The acidic attack dissolves the whole mass of the alloy, so that selective leaching is impossible, and large volumes of acid are consumed. This is a problem with the recovery of rare earths from neodymium-iron-boron magnets, since 70 wt% of the magnet alloy consists of iron.
The pyrometallurgical recycling of.
× VitalSource eBook VitalSource Bookshelf gives you access to content when, where, and how you want. When you read an eBook on VitalSource Bookshelf, enjoy such features as:. Access online or offline, on mobile or desktop devices. Bookmarks, highlights and notes sync across all your devices. Smart study tools such as note sharing and subscription, review mode, and Microsoft OneNote integration. Search and navigate content across your entire Bookshelf library.
Interactive notebook and read-aloud functionality. Look up additional information online by highlighting a word or phrase. This multi-author new edition revises and updates the classic reference by William G. Davenport et al (winner of, among other awards, the 2003 AIME Mineral Industry Educator of the Year Award 'for inspiring students in the pursuit of clarity'), providing fully updated coverage of the copper production process, encompassing topics as diverse as environmental technology for wind and solar energy transmission, treatment of waste by-products, and recycling of electronic scrap for potential alternative technology implementation.
The authors examine industrially grounded treatments of process fundamentals and the beneficiation of raw materials, smelting and converting, hydrometallurgical processes, and refining technology for a mine-to-market perspective - from primary and secondary raw materials extraction to shipping of rod or billet to customers. The modern coverage of the work includes bath smelting processes such as Ausmelt and Isasmelt, which have become state-of-the-art in sulfide concentrate smelting and converting. Key Features. Preface Preface to the Fourth Edition Preface to the Third Edition Preface to the Second Edition Preface to the First Edition Chapter 1. Overview 1.1. Introduction 1.2.
Extracting Copper from Copper–Iron–Sulfide Ores 1.3. Hydrometallurgical Extraction of Copper 1.4. Melting and Casting Cathode Copper 1.5. Recycle of Copper and Copper-alloy Scrap (Chapters 18 and 19Chapter 18Chapter 19) 1.6. Summary Chapter 2. Production and Use 2.1. Copper Minerals and Cut-off Grades 2.2.
Location of Extraction Plants 2.3. Price of Copper 2.4. Summary Chapter 3.
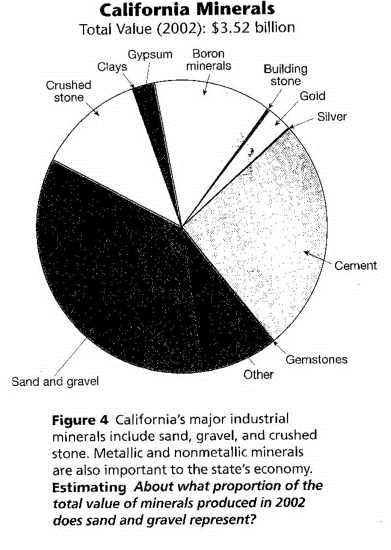
Production of High Copper Concentrates – Introduction and Comminution 3.1. Concentration Flowsheet 3.2. The Comminution Process 3.3.
Blasting 3.4. Crushing 3.5. Grinding 3.6. Recent Developments in Comminution 3.7. Summary Chapter 4. Production of Cu Concentrate from Finely Ground Cu Ore 4.1.
Froth Flotation 4.2. Flotation Chemicals (Nagaraj & Ravishankar, 2007; Woodcock, Sparrow, Bruckard, Johnson, & Dunne, 2007) 4.3. Specific Flotation Procedures for Cu Ores 4.4. Flotation Cells 4.5.
Sensors, Operation, and Control 4.6. The Flotation Products 4.7. Other Flotation Separations 4.8. Summary Chapter 5.
Matte Smelting Fundamentals 5.1. Why Smelting? Matte and Slag 5.3. Reactions During Matte Smelting 5.4. The Smelting Process: General Considerations 5.5.
Smelting Products: Matte, Slag and Offgas 5.6. Summary Chapter 6. Flash Smelting 6.1. Outotec Flash Furnace 6.2. Peripheral Equipment 6.3.
Flash Furnace Operation 6.4. Control (Fig. Impurity Behavior 6.6. Outotec Flash Smelting Recent Developments and Future Trends 6.7. Inco Flash Smelting 6.8. Inco Flash Furnace Summary 6.9.
Outotec Flash Smelting 6.10. Summary Chapter 7. Submerged Tuyere Smelting 7.1. Noranda Process (Prevost, Letourneau, Perez, Lind, & Lavoie, 2007; Zapata, 2007) 7.2. Reaction Mechanisms 7.3.
Operation and Control 7.4. Production Rate Enhancement 7.5. Teniente Smelting 7.6. Process Description 7.7. Operation (Moyano et al., 2010) 7.8.
Control (Morrow & Gajaredo, 2009; Moyano et al., 2010) 7.9. Impurity Distribution 7.10.
Discussion 7.11. Vanyukov Submerged-Tuyere Smelting 7.12.
Summary Chapter 8. Converting of Copper Matte 8.1.
Chemistry 8.2. Industrial Peirce–Smith Converting Operations 8.3.
Oxygen Enrichment of Peirce–Smith Converter Blast 8.4. Maximizing Converter Productivity 8.5. Recent Improvements in Peirce–Smith Converting 8.6. Alternatives to Peirce–Smith Converting 8.7.
Summary Chapter 9. Bath Matte Smelting 9.1. Basic Operations 9.2.
Feed Materials 9.3. The TSL Furnace and Lances 9.4. Smelting Mechanisms 9.5. Startup and Shutdown 9.6. Current Installations 9.7.
Copper Converting Using TSL Technology 9.8. The Mitsubishi Process 9.9. The Mitsubishi Process in the 2000s 9.10. Summary Chapter 10. Direct-To-Copper Flash Smelting 10.1. Advantages and Disadvantages 10.2.
The Ideal Direct-to-Copper Process 10.3. Industrial Single Furnace Direct-to-Copper Smelting 10.4. Chemistry 10.5. Effect of Slag Composition on% Cu-in-Slag 10.6.
Industrial Details 10.7. Control 10.8. Electric Furnace Cu-from-Slag Recovery 10.9. Cu-in-Slag Limitation of Direct-to-Copper Smelting 10.10. Direct-to-Copper Impurities 10.11. Summary Chapter 11. Copper Loss in Slag 11.1.
Copper in Slags 11.2. Decreasing Copper in Slag I: Minimizing Slag Generation 11.3. Decreasing Copper in Slag II: Minimizing Copper Concentration in Slag 11.4. Decreasing Copper in Slag III: Pyrometallurgical Slag Settling/Reduction 11.5. Decreasing Copper in Slag IV: Slag Minerals Processing 11.6. Summary Chapter 12. Capture and Fixation of Sulfur 12.1.
Offgases from Smelting and Converting Processes 12.2. Sulfuric Acid Manufacture 12.3. Smelter Offgas Treatment 12.4. Gas Drying 12.5. Acid Plant Chemical Reactions 12.6. Industrial Sulfuric Acid Manufacture (Tables 12.4, 12.5, and 12.6) 12.7. Alternative Sulfuric Acid Manufacturing Methods 12.8.
Recent and Future Developments in Sulfuric Acid Manufacture 12.9. Alternative Sulfur Products 12.10.
Future Improvements in Sulfur Capture 12.11. Summary Chapter 13.
Fire Refining (S and O Removal) and Anode Casting 13.1. Industrial Methods of Fire Refining 13.2. Chemistry of Fire Refining 13.3. Choice of Hydrocarbon for Deoxidation 13.4. Casting Anodes 13.5.
Continuous Anode Casting 13.6. New Anodes from Rejects and Anode Scrap 13.7. Removal of Impurities During Fire Refining 13.8.
Summary Chapter 14. Electrolytic Refining 14.1. The Electrorefining Process 14.2. Chemistry of Electrorefining and Behavior of Anode Impurities 14.3. Equipment 14.4. Typical Refining Cycle 14.5.
Electrolyte 14.6. Maximizing Copper Cathode Purity 14.7. Minimizing Energy Consumption 14.8. Industrial Electrorefining 14.9. Recent Developments and Emerging Trends in Copper Electrorefining 14.10. Summary Chapter 15. Hydrometallurgical Copper Extraction 15.1.
Copper Recovery by Hydrometallurgical Flowsheets 15.2. Chemistry of the Leaching of Copper Minerals 15.3.
Leaching Methods 15.4. Heap and Dump Leaching 15.5. Vat Leaching 15.6.
Agitation Leaching 15.7. Pressure Oxidation Leaching 15.8. Future Developments 15.9. Summary Chapter 16. Solvent Extraction 16.1. The Solvent-Extraction Process 16.2.
Chemistry of Copper Solvent Extraction 16.3. Composition of the Organic Phase 16.4. Minimizing Impurity Transfer and Maximizing Electrolyte Purity 16.5. Equipment 16.6. Circuit Configurations 16.7. Quantitative Design of a Series Circuit 16.8.
Quantitative Comparison of Series and Series-Parallel Circuits 16.9. Operational Considerations 16.10. Industrial Solvent-Extraction Plants 16.11. Summary Chapter 17. Electrowinning 17.1. The Electrowinning Process 17.2. Chemistry of Copper Electrowinning 17.3.
Electrical Requirements 17.4. Equipment and Operational Practice 17.5. Maximizing Copper Purity 17.6. Maximizing Energy Efficiency 17.7. Modern Industrial Electrowinning Plants 17.8. Electrowinning from Agitated Leach Solutions 17.9. Current and Future Developments 17.10.
Summary Chapter 18. Collection and Processing of Recycled Copper 18.1.
The Materials Cycle 18.2. Secondary Copper Grades and Definitions 18.3.
Scrap Processing and Beneficiation 18.4. Summary Chapter 19. Chemical Metallurgy of Copper Recycling 19.1. Characteristics of Secondary Copper 19.2. Scrap Processing in Primary Copper Smelters 19.3. The Secondary Copper Smelter 19.4.
Summary Chapter 20. Melting and Casting 20.1.
Product Grades and Quality 20.2. Melting Technology 20.3.
Casting Machines 20.4. Summary Chapter 21. Byproduct and Waste Streams 21.1.
Molybdenite Recovery and Processing 21.2. Flotation Reagents 21.3. Operation 21.4. Optimization 21.5. Anode Slimes 21.6.
Dust Treatment 21.7. Use or Disposal of Slag 21.8. Summary Chapter 22. Costs of Copper Production 22.1. Overall Investment Costs: Mine through Refinery 22.2.
Overall Direct Operating Costs: Mine through Refinery 22.3. Total Production Costs, Selling Prices, Profitability 22.4. Concentrating Costs 22.5. Smelting Costs 22.6. Electrorefining Costs 22.7. Production of Copper from Scrap 22.8.
Leach/Solvent Extraction/Electrowinning Costs 22.9. Profitability 22.10. Summary Index.
Professor William George Davenport is a graduate of the University of British Columbia and the Royal School of Mines, London. Prior to his academic career he worked with the Linde Division of Union Carbide in Tonawanda, New York. He spent a combined 43 years of teaching at McGill University and the University of Arizona. His Union Carbide days are recounted in the book Iron Blast Furnace, Analysis, Control and Optimization (English, Chinese, Japanese, Russian and Spanish editions). During the early years of his academic career he spent his summers working in many of Noranda Mines Company’s metallurgical plants, which led quickly to the book Extractive Metallurgy of Copper. This book has gone into five English language editions (with several printings) and Chinese, Farsi and Spanish language editions. He also had the good fortune to work in Phelps Dodge’s Playas flash smelter soon after coming to the University of Arizona.
This experience contributed to the book Flash Smelting, with two English language editions and a Russian language edition and eventually to the book Sulfuric Acid Manufacture (2006), 2nd edition 2013. In 2013 co-authored Extractive Metallurgy of Nickel, Cobalt and Platinum Group Metals, which took him to all the continents except Antarctica. He and four co-authors are just finishing up the book Rare Earths: Science, Technology, Production and Use, which has taken him around the United States, Canada and France, visiting rare earth mines, smelters, manufacturing plants, laboratories and recycling facilities. Professor Davenport’s teaching has centered on ferrous and non-ferrous extractive metallurgy.
He has visited (and continues to visit) about 10 metallurgical plants per year around the world to determine the relationships between theory and industrial practice. He has also taught plant design and economics throughout his career and has found this aspect of his work particularly rewarding. The delight of his life at the university has, however, always been academic advising of students on a one-on-one basis. Professor Davenport is a Fellow (and life member) of the Canadian Institute of Mining, Metallurgy and Petroleum and a twenty-five year member of the (U.S.) Society of Mining, Metallurgy and Exploration.
He is recipient of the CIM Alcan Award, the TMS Extractive Metallurgy Lecture Award, the AusIMM Sir George Fisher Award, the AIME Mineral Industry Education Award, the American Mining Hall of Fame Medal of Merit and the SME Milton E. Wadsworth award. In September 2014 he will be honored by the Conference of Metallurgists’ Bill Davenport Honorary Symposium in Vancouver, British Columbia (his home town).